Introduction
Over the past decades, excellent methods of water treatment have been developed to serve numerous industries and also the human life [1]. Membrane technology has been widely used for the purification and separation of toxic components from water, largely due to its simple operation, excellent selectivity and high permeability features. This technology is optimal for the removal of undesirable components and toxic ions in water treatment plants [2]. For this purpose, various membranes have been designed and successfully used, depending on the specific water treatment and purification purposes. Among them, nanofiltration (NF) has been remarkably successful, due to the low energy and cost, high permeability and flux rates, and simple operation [3]. In this context, the demands for new NF-membranes are steadily growing with the aim of removing hazardous, soluble pollutants and disease-producing ions, such as heavy metals from public water supplies.
Different polymeric materials are used for the fabrication of NF-membranes. Among them, polyethersulphone is well-known as an established material due to its perfect thermal stability, mechanical strength and resistance against chemicals [4]. Nevertheless, it has a hydrophobic surface and high tendency for fouling, which block the pores and eventually disrupts the membrane function [5, 6]. Specifically, the dysfunction results from the reduction in the permeation flux, rejection, membrane life span, and the increasing costs of production. However; fouling is the main obstacle in the performance of such membranes [4, 7]. Various efforts have been made to produce highly efficient membranes besides overcoming the fouling drawback [8]. In this context, surface modification is an effective method to improve the filtration and antifouling behavior of membranes by modifying their charges and surface roughness [7]. Hence, surface coating has emerged as a desirable strategy to physically modify membranes [5]. Through this approach, the membrane base is immersed into a solution to coat its surface with a thin layer of material without causing any chemical interaction [9].
Chitosan, a hydrophilic and charged polymer, is one of the highly studied materials used to coat the surface of membranes [10]. It is a polyamine saccharide, which is derived from chitin [11]. This biopolymer consists of functional OH and NH2 groups [12], which cause the hydrophilic effect for the separation of toxic ions and biomolecules [12]. Moreover, the perfect film-forming property, lack of toxicity, high mechanical strength, desirable adsorption property, and antibacterial features of chitosan make it an excellent candidate for membrane modification [10-13]. Other attractive points to mention are the high flux and perfect rejection rate in membranes that make them ideal filters, such as those with a thin layer of chitosan on their surface [14].
In contrast to the above-mentioned positive properties, the coating’s functional groups are hydrated in water and swell up, thus these membranes may not work well over long-term. To prevent this issue, a variety of nanopar ticles can be used to coat membranes [14]. Due to their size and structure, nanoparticles have been widely used to modify the membranes’ thin surface coating [15]. Utilizing hydrophilic nanoparticles, such as polyaniline to coat the membranes’ surface is necessary to cause significant hydrophilicity, high water flux and permeation of the substrate, and excellent ionic rejection [16]. Also, polyaniline nanoparticles are available at low cost, easy to synthesize and are non-toxic [16].
Aim of the study: In the current study, a nano-composite thin film was developed and coated on a virgin membrane of choice. The membrane’s coating material consisted of chitosan, polyaniline and polyethersulphone, interacted with ethylene glycol. The expected outcome was to promote significant antifouling and filtration of toxic metal ions from wastewater samples. The specific aim of this study was to investigate the physic-chemical, structural, antifouling and filtration characteristics of a virgin membrane, coated with a thin-film and with the ability to filter out chromium and copper ions from wastewater samples.
Materials and Methods
Materials: Polyethersulphone (PES) at a molecular weight of 58000 daltons was obtained from BASF (New Jersey, USA), and used as the membrane base. We utilized N,N-Dimethylacetamide (DMAC, 8712 g/mol, 0.94 g/cm3) and polyvinylpyrrolidone as the solvent and pore-former, respectively. These compounds were purchased from Merck Inc. (Darmstadt, Germany) Ethylene glycol was provided from Merck Inc. (Germany) and chitosan (Cs, Mw100.000-300.000, 90% deacetylated) was supplies from Across Inc., USA. Aniline (Mw93.13) from Merck Inc., and HCl (Mw36.46, 1.19 g/cm3) provided by Mojallali Co. (Tehran, Iran), and ammonium persulfate (Mw228.2) (Merck Inc., Germany) were used for the synthesis of polyaniline nanoparticles. All other chemical were obtained from a local agent for Merck Inc. (Tehran, Iran).
Polyethersulphone-based membrane and fabrication: The PES-based membrane was developed by a phase inversion method. For this purpose, PES (18 wt %) was dissolved in DMAC and 1% PVP and added to the solution to generate optimal pore structure. The prepared solution was stirred continuously for at least 7 hr to homogeneity. Then, the solution was kept at room temperature for 24 hr to ensure the air bubbles disappeared completely. The membrane development ended up by casting solution on glass plates, using proper applicator with a fixed 200 µm thickness. At the next step, the polymeric solution container was immersed in a water bath immediately. It was then kept in DI-water to remove excess solution for 24 hr at ambient temperature to complete dryness.
The thin-film membrane was fabricated by the coating method through networking with ethylene glycol. For this purpose, chitosan (0.3%, wt) was dissolved in 60 mL aqueous solution containing 5 mL HCl and stirred continuously for 40 min. The PES-based membrane was then dipped in the chitosan solution and sonicated for 15 minutes to complete homogeneity at room temperature. In another container, different concentrations of nanoparticles were mixed in water under stirring. The process was followed by dipping the membranes in the nanoparticle solution under constant stirring. Also, 1-mL ethylene glycol was added to all containers as a cross-linking agent. Then, 0.6 g potassium persulfate was added slowly to start the process. The solutions were sonicated for 30 min to achieve a homogeneous spread of the nanoparticles on the membrane surface. Finally, it was heated at 70°C for 90 minutes and was then washed with DI water. Table 1 shows the features of the membranes developed by this study.
Synthesis of polyaniline nanoparticles: The polyaniline nanoparticles were prepared by facile chemical polymerization method, based on the details as described in previous articles [17-19]. For this purpose, 3 mL aniline monomer was added to 50 mL 0.1 M HCl solution and sonicated for 15 minutes. Then, 6 g ammonium persulfate was added to solution drop-wise under constant stirring in an ice bath, as the reaction initiator. When the solution turned dark green, the polymerization was complete. The final product was washed with DI water and dried in an oven at 50ºC for 48 hr.
Membrane’s characterizations: To investigate the chemical structure, Fourier transform infrared spectroscopy (FTIR) was applied by a single beam spectrometer (Galaxy series 5000) at 400 to 4000 cm-1. The field emission scanning electron microscopy (FESEM, MIRA3, and TESCAN) were also employed to study the surface and cross sectional morphology of the membranes, which were coated with gold before examination.
The 3D-images were also used to study the surface roughness of the membranes. The images were taken under light microscopy and analyzed by FEMTO scan software, version 2.3.219 [20]. The surface hydrophilicity/wettability of the membranes was examined by measuring the water contact angle. The test was done by droplets of DI-water on the membrane surface. To minimize errors, the water contact angle was tested at five different random locations and the averages recorded. The membranes’ water content was studied as the weight difference between the dry and wet samples. This test determined the amount of absorbed or accommodated water in the membranes. To obtain the water content, the samples (in square shape) were first immersed in water for 24 hr at ambient temperature. Next, the wet membranes were kept between 2 filter papers to wipe the extra water on the surface and weighted immediately. Membranes were then heated in an oven at 60ºC until a constant weight was achieved, representing the dry membrane. The following Equation 1 was used to calculate the membranes’ water content [8]:
Where Wd and Ww are the weight of the dry and wet membranes (g), respectively. To obtain the flux and separation properties of the membranes, we used a stainless steel dead-end cell module with 11.94 cm2 effective areas connected with nitrogen gas. Initially, all membranes were soaked in deionized water at 5 bar pressure for 15 min. Each membrane was then pressurized with distilled water at 5 bar for 1 hr. The flux was defined as the amount of water passing through the membranes based on Equation 2, as shown below [7]:
.png)
Where Q is the volume of permeated water in liter, A is the membrane effective area in m2, t is the permeation time in hr, and J represents the flux in l/m2/hr.
The salt rejecting power of the membranes was analyzed with solutions containing sodium, chromium and copper sulfates. Subsequently, we examined the ability of membranes (rejection percentages) to remove the toxic elements (Cr & Cu) from the wastewater samples, using the following Equation 3:
.png)
Where Cp and Cf represent the ion concentrations in permeates and feed [7]. To estimate the antifouling potential of the membranes, a powdered milk solution was used, as a strong foulant at 8000 mg/L. We measured the permeation flux during filtration process before and after the powdered milk solution test, at five bar pressure for 30 minutes. The flux recovery ratio (FRR), total resistance (Rt), reversible resistance (Rr), and irreversible resistance (Rir) were also calculated based on Equation 4-7:
Where, Jw1, Jw2, and Jp represent the water permeation for virgin membranes, permeation of water for virgin membranes, and flux of powdered milk solution, respectively [7].
Results and Discussion
Membranes spectroscopy: The chemical structure of PES-based, thin-film membrane was examined, using FTIR spectroscopy, the results of which are shown in Figure 1.
The peaks at 1408, 1488, 1581/cm were assigned to aromatic bands and those at 1485/cm shows C-C to stretch. Also the peaks at 1169 and 1105/cm were assigned to C=SO2=C symmetric stretch and bands at 1225/cm represented the C-O-C stretch (Figure 1). The FTIR images indicate that the bands linked to C-N at 1105, 1255/cm and 1593/cm are benzene ring. The broad peak near 3000-3600/cm is attributed to -OH symmetrical vibration of hydroxyl group, which is believed to be a functional group in the chitosan molecule. The vibration of amine groups around 3500/cm is illustrated simultaneously. Moreover, the functional group, NH2, represents the peak at the 1600-1700/cm range [20-22].
Membrane morphology: The SEM images of the membrane are illustrated in Figure 2.
Figure 2. Surface electron micrographs of the membranes’ cross-sectional areas
The asymmetrical structure, dense layer and porous sub-layer were noted in the SEM images. The active layer is denser and contains small pores. As noted in the SEM images, accumulation of nanoparticles on the surface is found at high nanoparticle ratios. The pores were cylindrical and stretched longitudinally in all membrane samples. In addition, the thickness of the active layer increased by raising the quantity of polyaniline nanoparticles. Usually, increases in density and thickness of the upper layer may lead to a decline in flux. However, it can improve the selectivity and filtration properties of the membrane.
The membrane’s SEM images are shown in Figure 3.
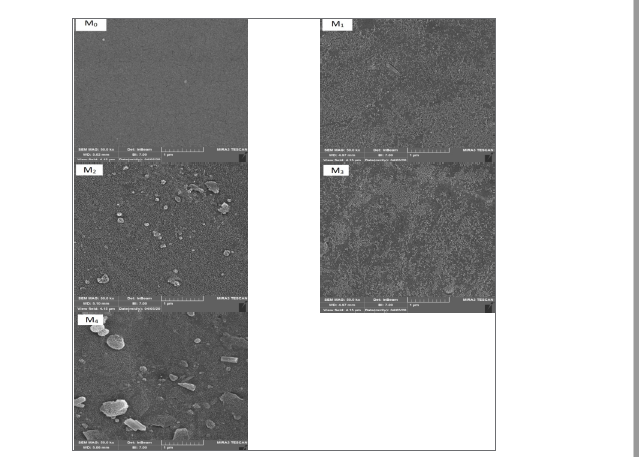
Figure 3. The surface SEM images for the fabricated membranes: PES-based and chitosan-polyaniline/PES thin-film membranes
Based on the findings, the surface underwent changes after the incorporation of nanoparticles. At medium (M2) and high (M4) concentration of nanoparticles, the accumulation occurred while for the M3 membrane, it retained its relative uniform surface. This may be due to optimal concentration of nanoparticles at the ratio that filled the pores and formed a uniform layer on the membrane’s surface.
The images produced by energy dispersive X-ray spectra (EDX) mapping of nitrogen for the chitosan-polyaniline layer is shown in Figure 4, confirming its formation (light spots).
To observe the surface roughness of the membrane, 3D-surface images were taken and examined. The smoother surfaces promoted better anti-fouling properties [23, 24]. The calculated average roughness (Ra) of the membrane is illustrated in Figure 5.
As seen in that Figure, the smoother surface on M1 is due to the pores and valleys filled by the formation of the layer on the virgin PES-based membrane. The average roughness decreased from 6.127 nm in PES-base membrane to 2.387 nm in M1. The increase in the surface roughness to 4.331 nm for M2 (CS-PANI 0.25/PES) could be linked to the agglomeration of nanoparticles. Also, there was an increase in the surface heterogeneity, which is likely due to the incorporation of polyaniline nanoparticles, resulting in greater number of peaks on the membrane’s surface [25]. The decline in the surface roughness for M3 and M4 may be associated with the frequency of nanoparticles at higher concentration that caused a uniform surface by filling the pores and valleys.
Water contact angle and content: The water contact angle measurement evaluates the membrane’s surface hydrophilicity/wettability, the results of which are presented in Figure 6.
As noted in that Figure, after applying chitosan to the surface of virgin PES-based membrane, the water contact angle declined from 59.5° to 55.3° due to the hydrophilic characteristic of chitosan. The increase in the surface hydrophilicity of M2 and M3 is also due to the hydrophilic functional groups of polyaniline. The increase in the contact angle of M4 membrane is linked to the agglomeration of nanoparticles, which leads to a decrease in the active surface area along with the availability of hydrophilic functional groups.
Based on the evidence shown in Figure 7
the membrane’s water content was enhanced by introducing chitosan and polyaniline nanoparticles up to 0.25 wt% from 67.76% for PES-based to 82.77% for M2 (CS-PANI 0.25%)/PES. After that point, it began to decline again at higher additive concentration. The increase in the water content is likely related to the hydrophilic nature of both the nanoparticles and chitosan. Moreover, the rise in the number of pores and spaces tend to enhance the presence of water molecules in the membrane. A reduction in these features could be explained by the membrane pores filling at higher polyaniline concentration. This event decreases the water content and less uniform distribution of the nanoparticles [25].
Water flux: The surface hydrophilicity or morphology of membrane can affect the water flux. This is directly associated with the increased hydrophilicity and surface smoothness, both of which improve the ability to absorb water molecules through the membrane [26]. As shown in Figure 8,
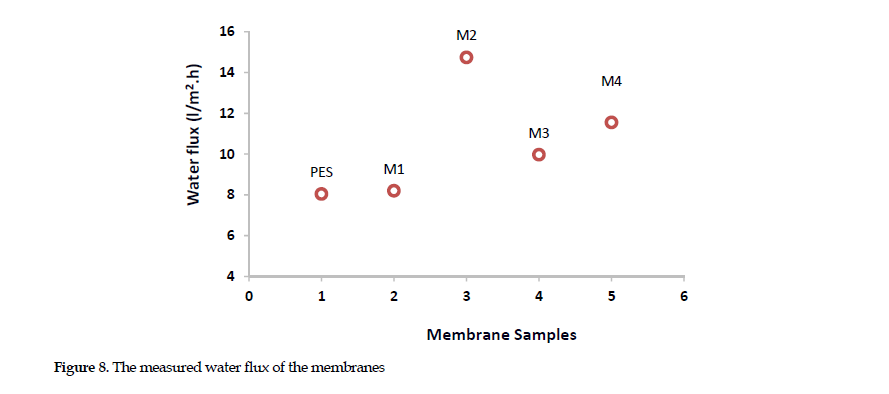
the water flux for M0 measured around 8.04 (l/m2/h) that was less than those for all of the modified membrane. This may be linked to the surface becoming more hydrophobic. Chitosan plays an effective role in improving membrane’s water flux. The functional group in chitosan molecule helps the surface pass more water molecules through the membrane. By adding polyaniline nanoparticles to M2 membrane, its pure water flux increased sharply. This increment could be attributed to the greater hydrophilicity of the surface and the high water content in M2 sample that increased the pure water flux to >14.74 l/m2/h. The decrease in water flux for M3 may be due to a reduction in free spaces in the membrane by nanoparticle accommodation, which reduced the water flux down to 9.97 l/m2/h. At high concentration of polyaniline nanoparticles, the rise in the water flux up to 11.55 l/m2/h for M4 sample may be due to the propagation of cracks and fissures, which occurred at high nanoparticle ratios. This change in turn facilitated the passage of water molecules through the membrane.
Salt rejection: To further assess the membrane performance, the salt rejection was studied (Figure 9).
Based on the findings, the sodium sulfate rejection declined by 61% and 54%, respectively, for PES-based and M2 membrane samples. The decline in the charge density and probably the nanoparticle agglomeration might have enhanced the salt rejection. The M3 membrane with the 0.5 wt% polyaniline nanoparticle had the highest salt rejection rate compared to other samples. The results demonstrated that the lowest water contact angle and dense surfaces for M3 and M4 increased their salt rejection rates [23, 24]. Further, the SEM images provided evidence in support of the increased thickness of the active layer, which was also responsible for a better salt rejection.
Removal of Toxic Heavy Metals: Based on the data presented in Figure 10,
from among the samples, the M3 and PES-based membranes were used for the study of the removal of the toxic ions, since they had shown optimal performance. The results showed high potential of thin-film membrane for the removal of chromium and copper ions from the wastewater samples, with the performance approaching over 86% and 84%, respectively, for the two membranes. Chitosan is one of the best adsorbent materials and is a biopolymer that improves the adsorption of heavy metals due to its repulsive forces. This biopolymer improves the ionic interaction with the membrane surface, due to having amine and hydroxyl functional groups. Introducing polyaniline also promotes the toxic ions rejection. Also, the NH group in this compound provides more active sites for the adsorption of heavy metal ions. The active sites and metal ions form complexes, which restrict their passing through the channels. The electrostatic interaction of amine functional group provides more active sites toward the adsorption of heavy metal ions.
The mapping of EDX analysis for Cr and Cu is presented in Figure 11.
As evident by the images, the adsorption behavior of thin-film membrane was a dominant factor in favor of heavy metal ions removal. Also, the greater amount of Cr removal compared to that of Cu was attributed to the high adsorptive capacity of this element (Figure 11).
Antifouling Properties: In order to explore the antifouling properties, we examined the flux recovery ratio (FRR) and fouling resistance parameters, including total fouling resistance (Rt), reversible fouling ratio (Rr) and irreversible fouling ratio (Rir). The results showed excellent anti-fouling properties for the thin-film membrane. The flux recovery ratio (FRR) measured >94.65% for M3 whereas it was >79% for PES-based membrane (M0). The data confirmed the superior performance of chitosan-polyaniline with respect to the hydrophilicity. The high fouling effect occurred for M0, because of the hydrophobic nature for this sample. The results also demonstrated that the irreversible resistance for M3 declined significantly (Figure 12).
This is attributable to the self-cleaning property of the membrane, which was modified by chitosan or polyaniline. Generally, utilizing nanoparticles and the fabrication method were the two effective factors that enhanced the antifouling ability of the novel membrane. Basically, the hydrophilic surface had a greater tendency to absorb water molecules compared to simple the surfaces. The water molecules form a thin layer on the surface act as an obstacle against the penetration of foulants, thus preventing bondage with the surface.
Conclusions
This study developed a novel thin-film nano-composite membrane, coated with chitosan and polyaniline for the removal of toxic heavy metal ions from wastewater. The FTIR spectra analysis of the data clearly exhibited the formation of a thin-film layer on the membrane. The surface and cross sectional SEM images showed the formation of a dense layer on the membrane’s surface. The 3D-images demonstrated that the surface roughness declined by increasing the polyaniline component in the surface layer. The surface wettability enhanced by the incorporation of polyaniline nanoparticles. The water flux improved to 8.04 L/m2/hr for the virgin membrane and up to 14.74 L/m2/hr for the thin-film samples. The sodium sulfate rejection rate was 61% for the virgin PES-based membrane whereas it was >67% for the novel thin-film nano-composite models. The results provided excellent evidence in favor of the anti-fouling properties of the novel membrane. Also, the FRR was over 94.65% for the thin-film membrane, while the value was 79% for the virgin membrane. The magnitude of chromium and copper ions removal by the thin-film membranes was over 86% and 84%, respectively, while the similar values for the virgin PES-based membrane were ~53% and ~51%, respectively.
Ethical Considerations
Compliance with ethical guidelines
There were no ethical considerations to be considered in this research.
Funding
This study was financially supported by Arak University, Arak, Iran.
Authors' contributions
Conceptualization and Supervision: Sayed Mohsen Hosseini; Study design and Methodology: Samaneh Koudzari Farahani and Sayed Mohsen Hosseini; Investigation: Sahar Karami, Zahra Jiriaei Sharahi and Samaneh Koudzari Farahani; Data collection: Sahar Karami and Zahra Jiriaei Sharahi; Data Analysis: Sahar Karami, Zahra Jiriaei Sharahi and Samaneh Koudzari Farahani and Sadra Solhi; Materials: Sadra Solhi; Literature review and writing draft: Sahar Karami; Critical review: Sadra Solhi and Sayed Mohsen Hosseini.
Conflict of interest
The authors declared there is no conflicts of interest.
Acknowledgements
Authors gratefully acknowledge Arak University for providing the financial support.
References