Intrpduction
Over the past decade, the environmental pollution and the severe shortage of water resources have grown considerably. It is very clear that seawater desalination is considered as a worthy method of regenerating freshwater suplies for public consumption [
1, 2]. In recent years, advanced functional ion exchange membranes (IEM) prepared with nano-particles have provided an excellent opportunity among various fields in industries and human life, for the separation of various types of liquid and gas contaminations [
3, 4]. Membrane-based seperations have been developed rapidly to generate clean water from various waste resources due to their greatly promising advantages [
5, 6].
Reverse osmosis (RO) technology has received much attention globally, among all other water desalination methods [
7]. However, recently RO developement has become limited due to the environmental problems arising from the resultant concentrates [
8]. Alternatively, electrodialysis (ED) has been preferred for the treatment of salty wastewaters, in which greater dilute brackish water is produced from the efficiency perspective, as an appropriate choice. The main reasons are its non-polluting and environmental friendliness compared to RO and thermal methods [
9, 10, 11, 12]. The ED and membrane deionization methods utilize ion exchange membranes to produce fresh water, involving materials with charged groups. Developing an effective IEM requires improved seperation properties, such as permselectivity, ionic conductivity and chemical stability [
13].
Nanocomposite ion exchange membranes are prepared by incorporating particle additives into polymeric membranes. These products have demonstrated considerable advantages for their mechanical strength and seperation charactristics, compared to the homogeneous counterparts [
14]. Recently, typical additives include carbon nanotubes [
15], silica [
16], graphene-based nanomaterials [
17], and copper ferrit [
18]. However, more recent studies, using IEM for wastewater desalination, have been concerned with the rational design of nanopore memranes. As indicated by a number of recent studies [
19, 20, 21, 22], the most ion diameters in salty wastewaters vary from 0.4 to 0.9 nm [
17], while the size of small organic molecules ranges 1−2 nm. In this context, the optimal size of membrane nanopores should be 0.9-1 nm to reach a high permselectivity for salt ions and organic molecules.
Further, utilizing nanoparticles at higher percentages can result in undesireable challenges, which may diminish the electrochemical characteristics of the resultant IEM [
19, 20]. In recent years, application of polydopamine (PDA) has recieved significant attention in the process of membrane modification [
21, 22]. Dopamine monomer is freely oxidized and polymerizes to create a crosslinked PDA [
23]. An earlier study [
24] has found that surface modification of membranes based on polyethylene (PE), polyvinylidene fluoride (PVDF) and polytetrafluoroethylene (PTFE) with polydopamine (PDA) may increase their hydrophilicity [
21]. Also studies have demonstrated that the interspaces between the accumulated PDA nanoaggregates can make nanopores sized 0.5 to 2 nm, in which transport mechanism can be controlled by the formation of PDA membranes [
24].
Aim of the study: This study aimed at developing a novel electrodialysis method to remove toxic heavy metals, such as copper and chromium, from wastewaters. We used polyvinylchloride (PVC) due to its desireable properties, mainly the chemical and biological resistance, and its easy processing.
Novelties of the study: Since PVC membranes show low permeability and high selectivity [
25], PDA may control and modify the seperation properties, improve the permeability and selectivity of PVC-PDA-CEMs. It can be obtained by the incorporation of such materials, as carbon nanofiber (CNF) to achieve a novel doublelayer cationic exchange Membrane (DLCEM). Other advantages of filtration with CNF membranes are attributed to their porous and interconnected structure, large surface area to volume ratios, and the submicron pore sizes. These features allow cationic exchange membranes to be permeable with superior filtration efficiency [
26].
Materials and Methods
Materials: All solvents and reagents were commercially available and used per the suppliers’ instructions. Polyvinylchloride (PVC; S-7054) was purchased from BIPC (Tehran, Iran), and used as a polymerbinder. Tetrahydrofuran (THF; 72.11g/g mol) was utilized as the base solvent. Dopamine hydrochloride and carbon nanofiber (CNF), used as the nanofiller, were purchased from Sigma-Aldrich. (Darmstadt, Germany).
Preparation of doublelayer cationic exchange membrane: The cationic exchange membranes (CEM), used as the substrate, were prepared by casting solution procedure. The PVC polymer, used as the main body,was dissolved in THF (1:20; w/v). Next, powdered resin particles (Amberlyst 15, >1.7 meq/g; Merck Inc., Germany) was added to the polymer solution at equal ratio (w/w). To break up the particle aggregates evenly, the mixture was sonicated for 90 min on an ultrasound apparatus (Parsonic, 28 KHz; Tehran, Iran). The solution was then casted on glass plates and were left at room temperature to complete dryness.
To make the ultimate modified membranes, dopamine hydrochloride powder and varying ratios of CNF, as the filler, were dissolved in 30 mL deionized water and sonicated for 2 hr. See details in
Table 1.
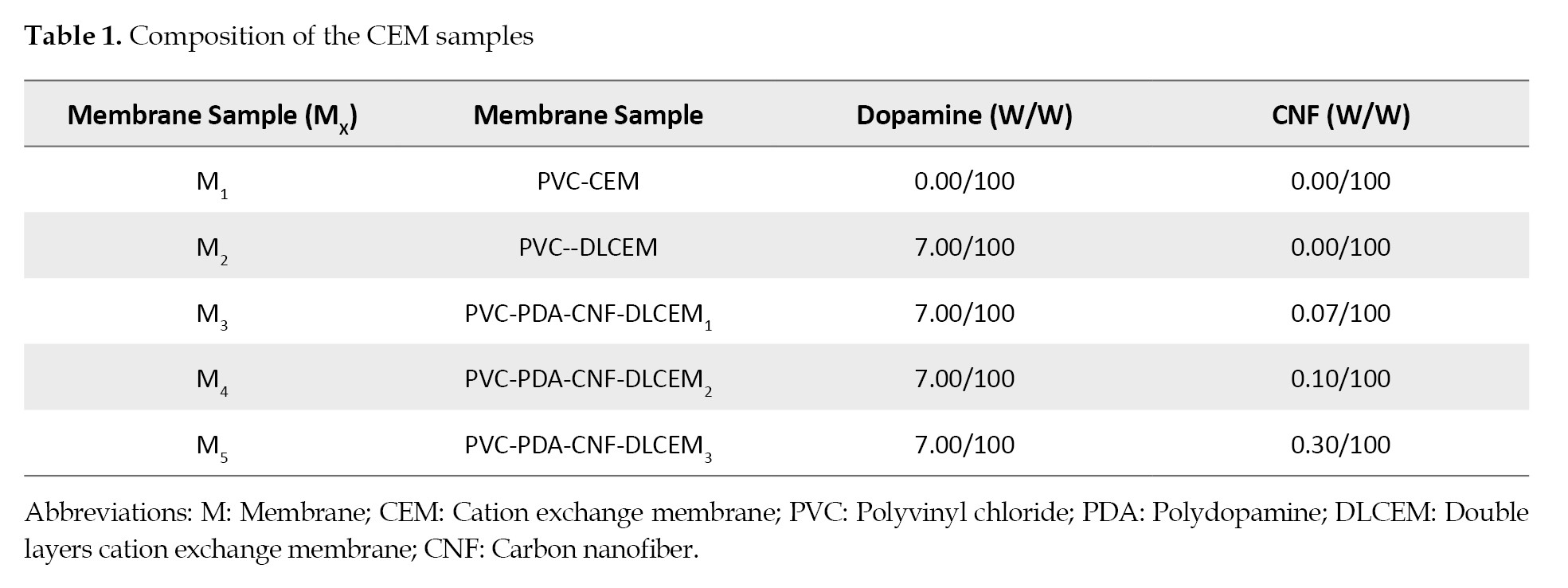
The clumps of PVC-CEMs with the area of 12.56 cm2 were prewetted in deionized water for 1 hr, and immersed into the prepared solution at pH 10 for dopamine polymerization on the membrane surface. Also, a few drops of galic acid (GA) was added to the solution as the crosslinking agent. The reaction continued for 3 hours at 90°C. Finally, the surface modified DLCEMs were rinsed with deionized water to clean the surface from the alkaline substances.
Membrane characterization: The surface morphology, structure and uniformity of the CEMs were examined under electron microscopy (FESEM, model: MIRA3TESCAN-XMU; Razi Foundation, Tehran, Iran). Also, the samples were chemically characterized by a single beam Fourier transform infrared spectrophotometer (FTIR, Galaxy series 5000). To evaluate the surface hydrophilicity, hydrophobicity, photocatalystic effects and surface self-cleaning of the membranes’ contact angel, these features were measured in deionized water at room temperaure.
Water content: The samples were dissolved in deionized water for 24 hr to measure the water uptake (10-4g), using an OHAUS apparatus (New Jersey, USA). The membranes’ wet weight (Wwet) was determined after removing the extra water droplets on a Wattman paper filter. Wet membranes were dried in an oven at 70°C for 4 hr, after which the water content was determined based on
Equation 1 as follows [
27, 28]:

Heavy metal ions removal The performance of the membranes for heavy metal ions removal was examined by an ED test cell in three parts. The concentrations of and cations were assayed in the aqueous solutions of CuSO4 and CrSO4, by atomic emission spectroscopy. The residual concentrations of the cations were used to estimate the percentage of the ions’ separation. The pH of the feed was set at 7. A simple schematic illustration of the ED setup is shown in
Figure 1.
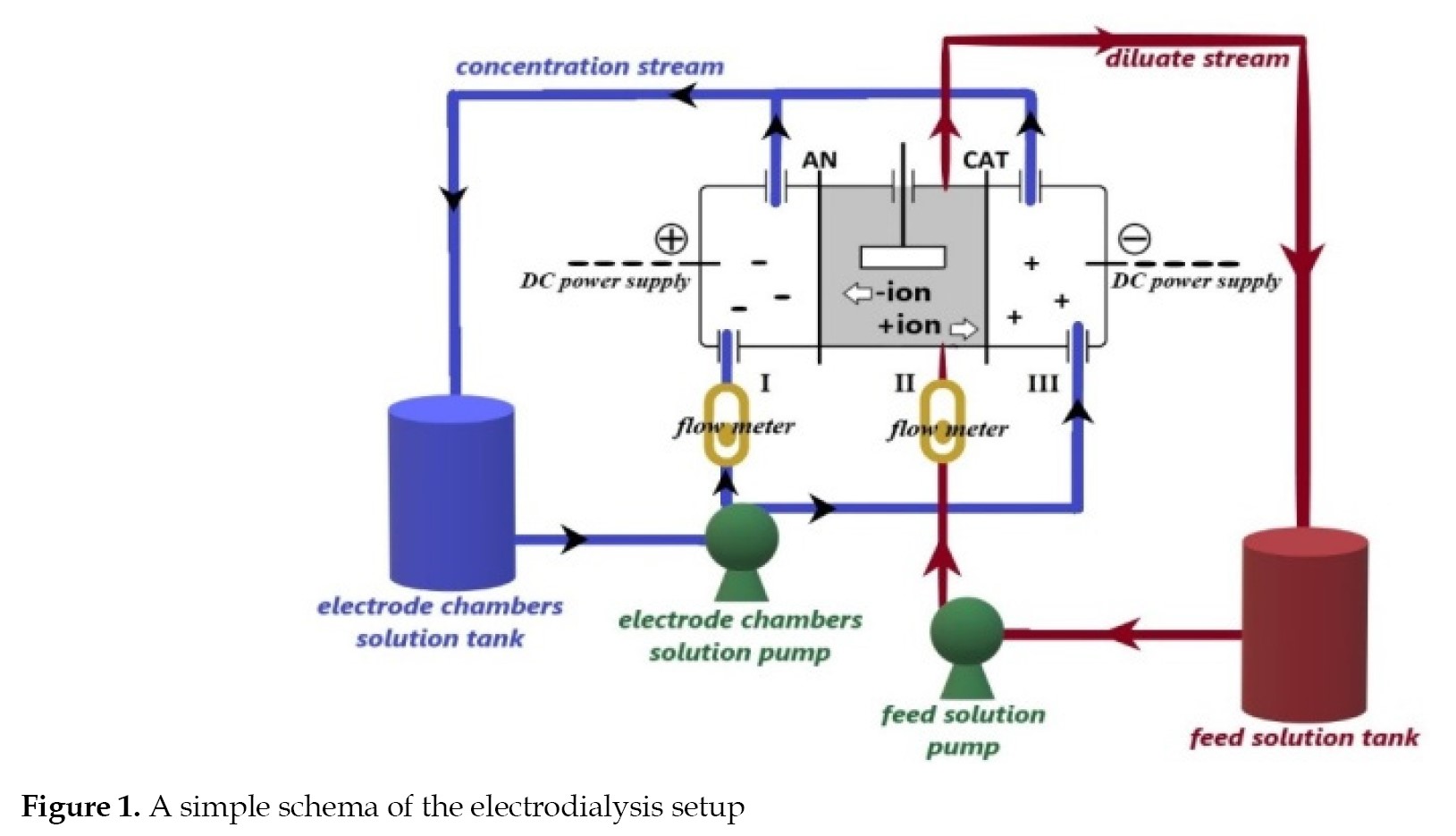
Measurement of ionic flux: To measure the ionic flux, a sodium chloride solution was used in test cells with platinum electrodes, with the terminals connected to direct current. The ionic flux in the membranes was measured at different pH settings of the compartments [
29, 30] .
Transport number and permselectivity: The current fraction carried by a special ion is considered as the transport number for that ion, which was determined based on
Equation 2 as follows:
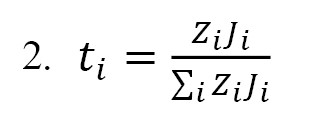
The above equation was also applied to determine the mean dynamic transport number (MDT) of the ions under study. This parameter was compared with static ones derived by Donnan potential. The permselectivity as assessed based on the counter ions’ migrations in membrane, using
Equation 3 as follows:
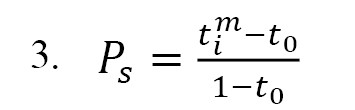
Where is the transport number of each counter ion [
30, 31].
Electrical resistance: The membranes were placed in a cell contaning electrolyte solution to determine the initial electrical resistance (R1). Then, the second resistance (R2) was measured, utilizing the apparatus without a sample. Finally, the membrane resisitance was determined, using the following
Equations 4 and 5:
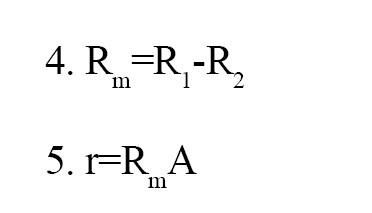
Where “r” is the real resistance and “A” is the surface area.
Rsults and Discussion
Characterization of the membranes
Morphological study:
Figure 2 illustrates the FTIR spectra of PVC-PDA-DLCEM membranes.
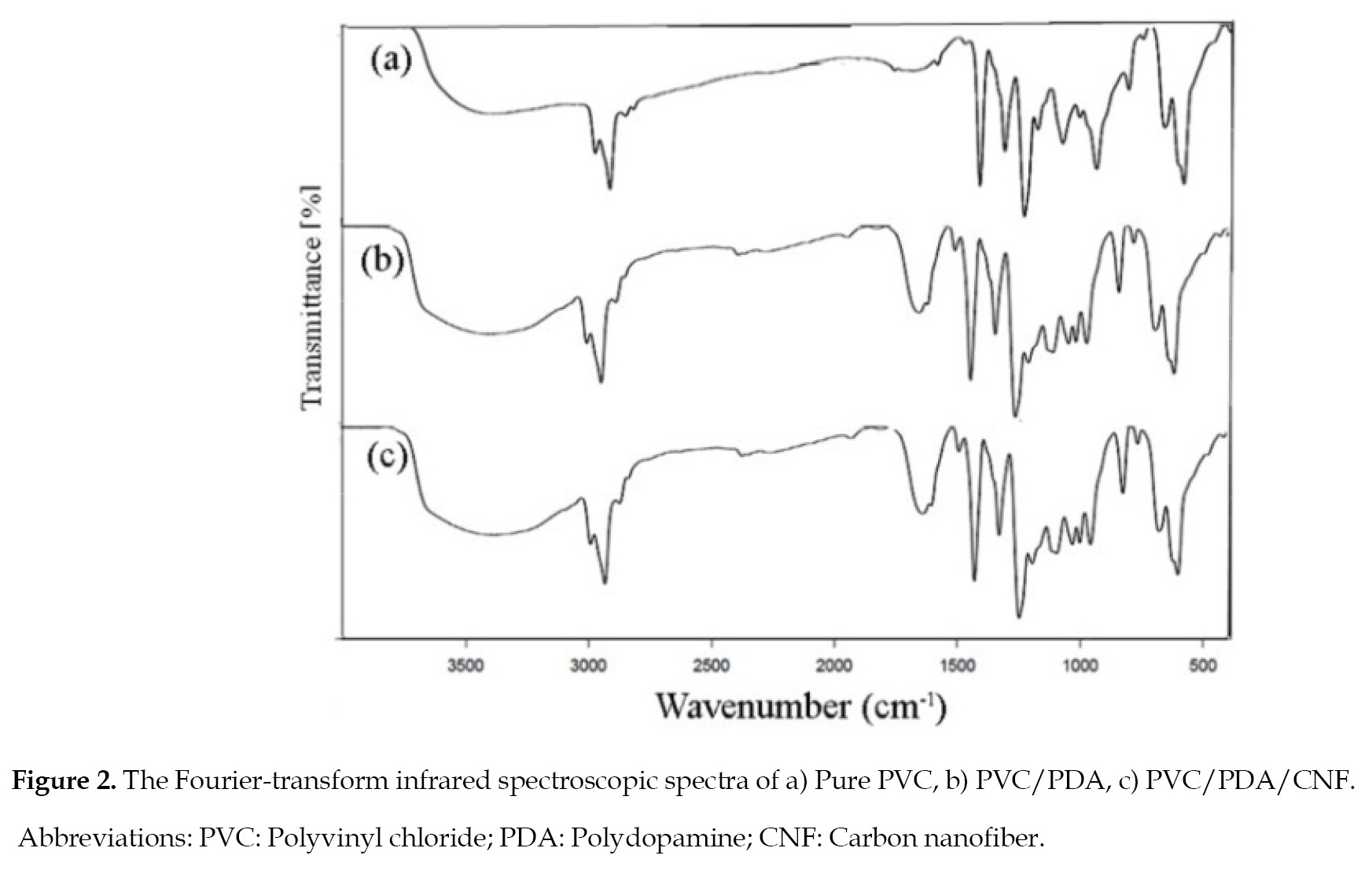
The spectrum for each sample was prepared to better describe the analysis of bonding fomation. The results indicated that the bonds at 3200-3500/cm were attributeable to the OH and NH groups in the modified membranes, and the presence of polydopamine on the surface. The peaks identified near the 1500 cm-1 and 1600 cm-1 represent the dopamine in the membranes, and denote the aromatic rings in its molecule. Also the peak at 1636 cm−1 is attributed to the OH symmetrical stretching vibration [
32, 33], and the peak at 1197.69 cm-1 is related to the carbon-chloride bonds in the PVC. The carbone-nitrogen bonds in the dopamine provide for the partial inter-chain interactions [
34]. It sould be noted that the CH2 and CF2 groups in PVC are characterized in 1330–1429 cm−1 and 1117–1250 cm−1 areas of the plots, respectively [
35, 36].
The FESEM images illustrated in
Figures 3 and
4 define the structural homogeneity, unifomity and integrity of the double-layer membranes with surface modifications, and incorporated additives.
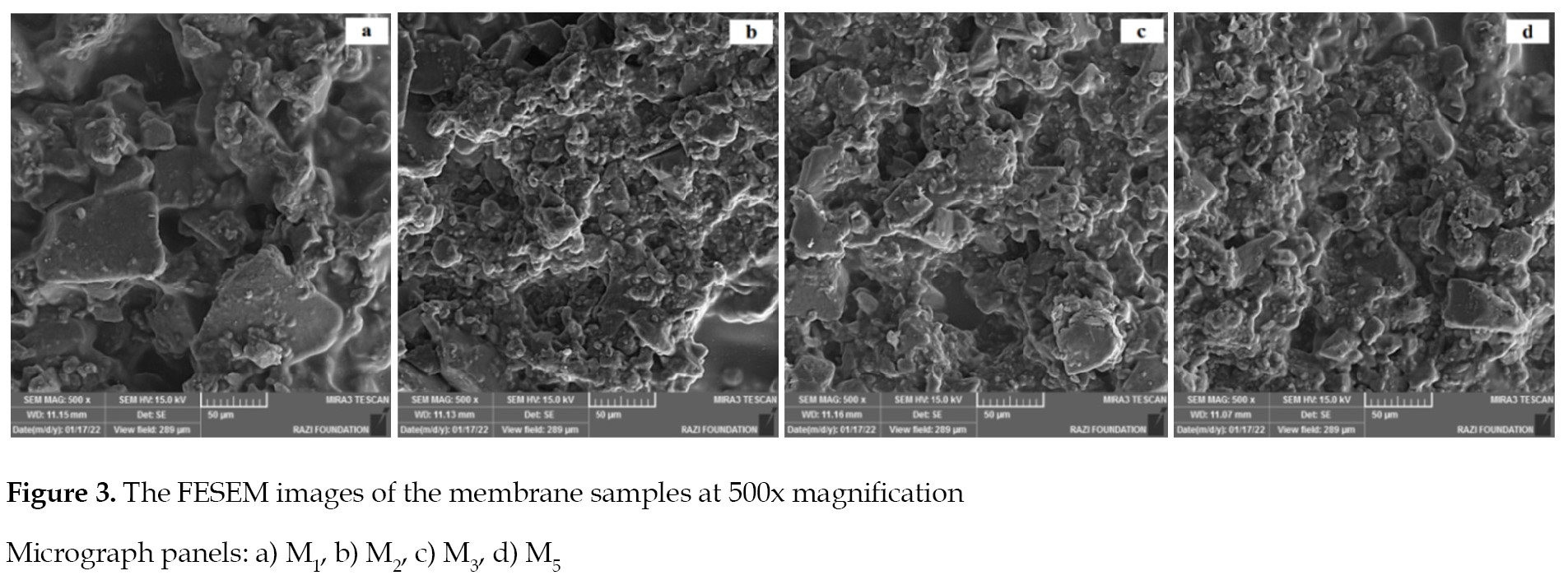
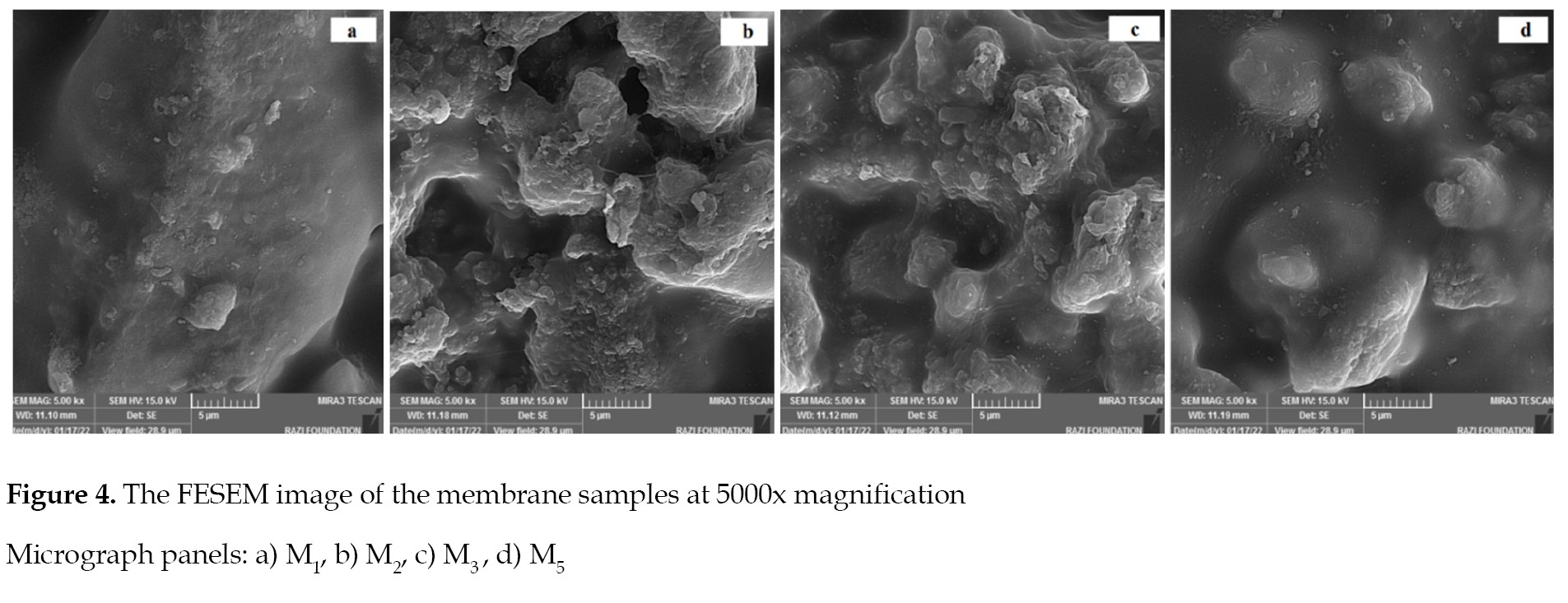
The resin particles and CNF additives were uniformly distributed on the membranes’ surfaces. It is worth noting that the incorporation of nanofibers into the membranes casting solution (
Figure 3 C,
M3 sample) caused the formation of cavities and voids. These occurred due to the sudden appearance of CNF additives and the increased nanofibers loading ratio up to 0.3% of the weight. This led to the development of sample, with a high concentration of CNF additives in the casting solution, which improved the integrity of the nanofibers’ agglomeration (
Figure 3 D).
Figure 4 represents the increase in surface roughness of the membranes modified with dopamine, which had negative effect on the antifouling property [
37]. In this study, it was noted that the most optimal loading ratio of dopamine was achieved by a correlation between surface hydrophilicity and roughness.
Water contents:
Table 2 presents the water contents of the membranes we developed, and the effects of incorporating PDA and CNF in them.
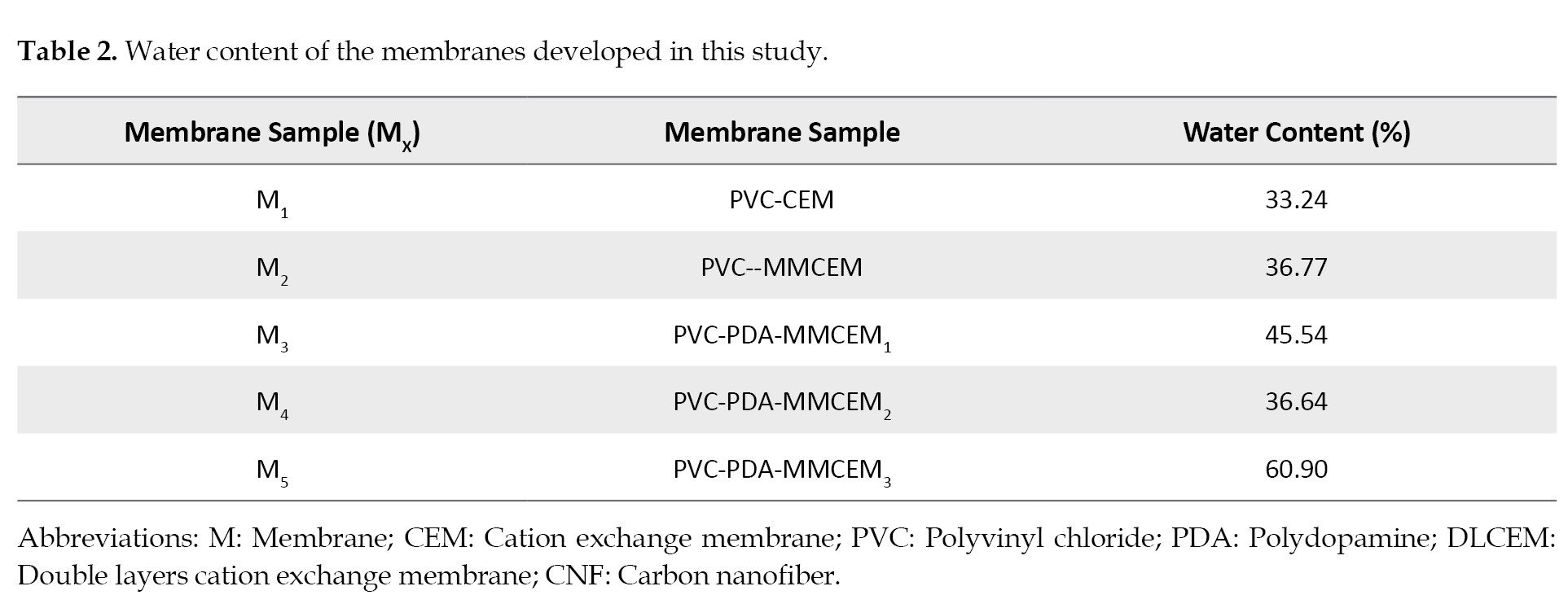
The increasing trend of water uptake may be related to the hydrophilic nature of PDA and nanofibers versus the hydrophobicity of the membrane surface [
38]. Results in
Figure 3 indicate that a rise in the nanoparticle concentration in the membranes icreases their water content. Thus, it may be concluded that the increase in the structural heterogeneity of the membranes may be linked to the presence of nanofibers (
Figure 3) [
39]. By further increasing the amount of the CNF nanofibers from 0.07% to 0.1%, the membranes’ water content showed a declining trend. It is likely that adding similar materials to the membranes’ matrix may lead to further decline in the water uptake capacity [
40].
Removal of heavy toxic metals: As shown by the data presented in
Figure 5, both M2 and M3 membranes had a greater capacity for the removal of Cu2+ and Cr2+ compared to M1.
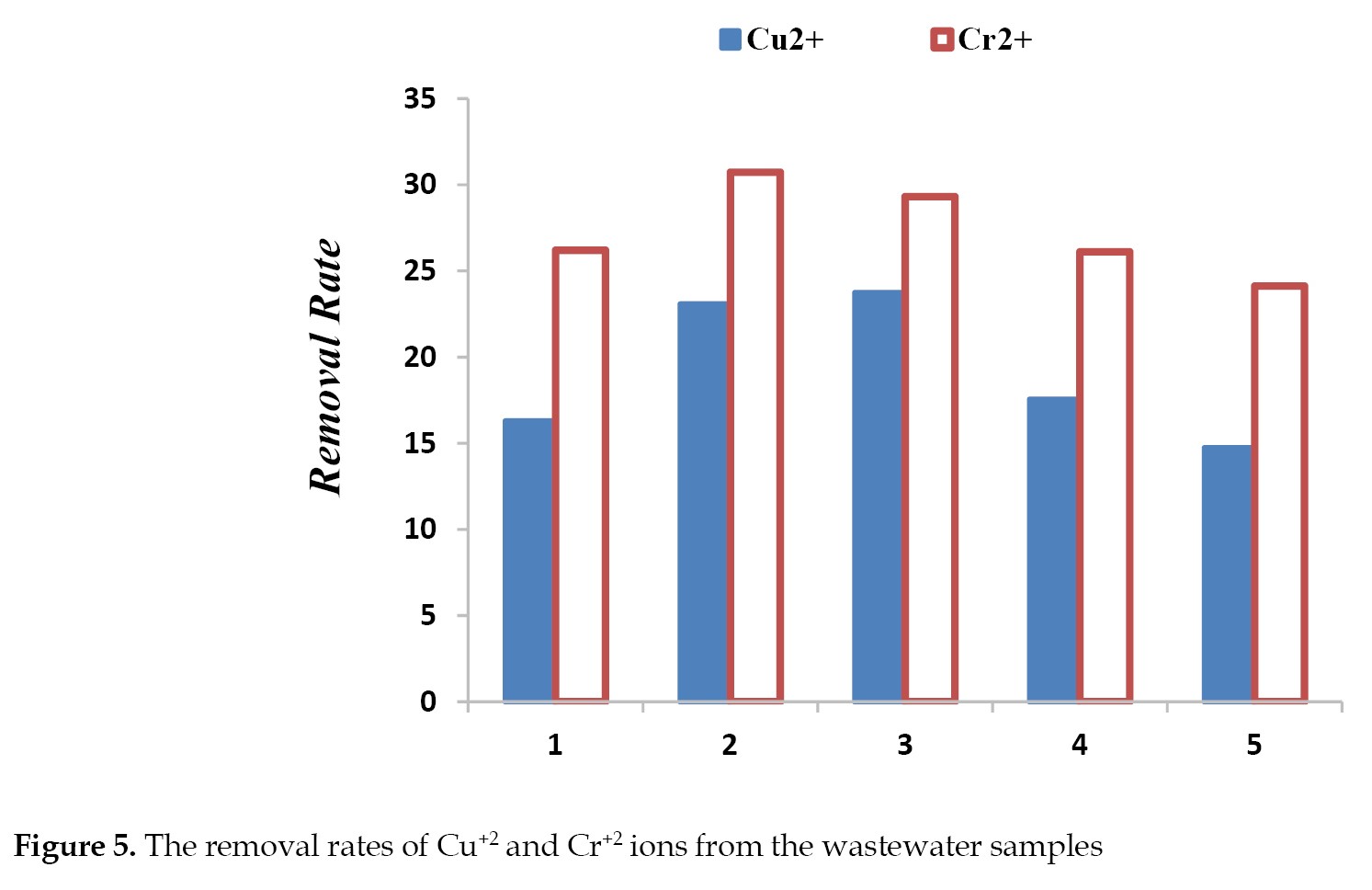
The higher efficiency of the membranes in the removal of the toxic ions, compared to M1, may be due to the greater hydrophilicity of PDA surface modification, and presence of –NH and –OH groups. Also, the increased porosity of the memberanes due to incorporating nanofibers, improves their ability to eliminate the toxic heavy metal ions from the wastewater samples. The electrostatic exclusion of the toxic ions by the negatively charged membranes’ surface is another reason for the observed improvement in their efficiency [
41,
42].
As shown in
Figure 5, with further increase in the nanofiber loading ratio of the membranes, the capacity to uptake and began to decline. This change in behavior may be due to the declining trend in the water content, seconday to a higher CNF loading ratio (
Table 2). Also, the nanofibers agglomeration led to lower porosity at the site due to a lower adsorption behavior [
34,
43]. Further, a higher ionic valence was likely to cause the greater ions repulsion from the membrane surface.
The elimination of the toxic ions increases by a rise in the valence of co-ions. Since the removal of the heavy metal ions improves with changes in the hydrated radius and charges of the anions, they lead to lower ions diffusion into the nanofibers and membrane surface. Indeed, the ionic removal capacity improves with an increase in the valence of co-ions and hydrated radius, because the ions repulsion occurs better at higher ionic valences. Also a high hydrated radius combined with lower ionic radius lead to ions lower diffusion into the nanoparticles and membranes surface. Therefore, Cr2+ with a higher hydrated radius and lower ionic radius than those of the Cu2+ is believed to have a higher removal capacity for ions than does Cu2+. This is likely to be caused by the steric hindrance effects and the ions difficulty in passing across nanoparticles [
41,
44].
The permselectivity: As reflected by the data in
Table 3, lower permselectivity for compared to the unmodified membrane , can be attributed to the enhanced water content, which may lead to a decline in the co-ions percolation.
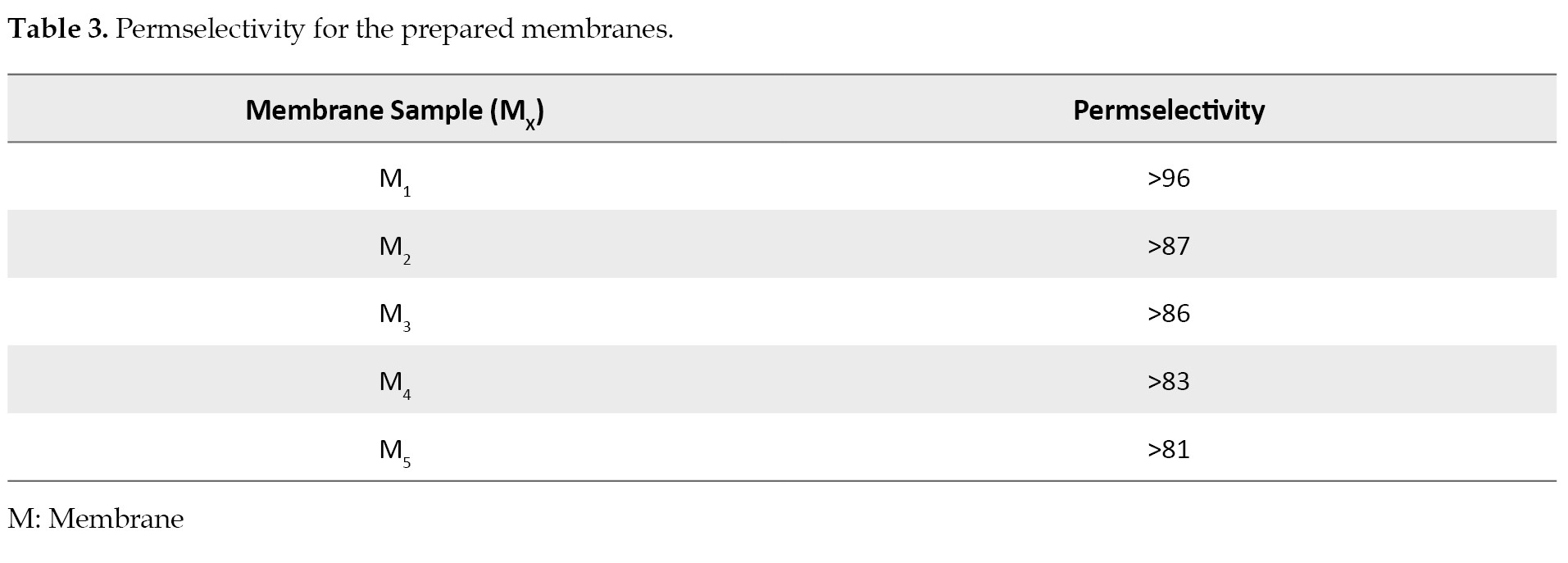
Under such conditions, greater amounts of water uptake can facilitate the ionic passage through the pathways [
18]. With an increase in the CNF concentration in the casting solution, the permselectivity remain almost constant, although has shown a greater reduction compared to . This behavior may also be attributed to the water content decline, as reflected in
Table 2. The filling of ion pathways by the CNF can narrow down the channels. Thus, the ionic agent groups transcend ion percolation, which may finally result in an increase in permselectivity or at least keep them constant.
Ionic permeability and flux: As illustrated in
Figure 6, membrane surface modification with PDA increased both the ionic permeability and flux.
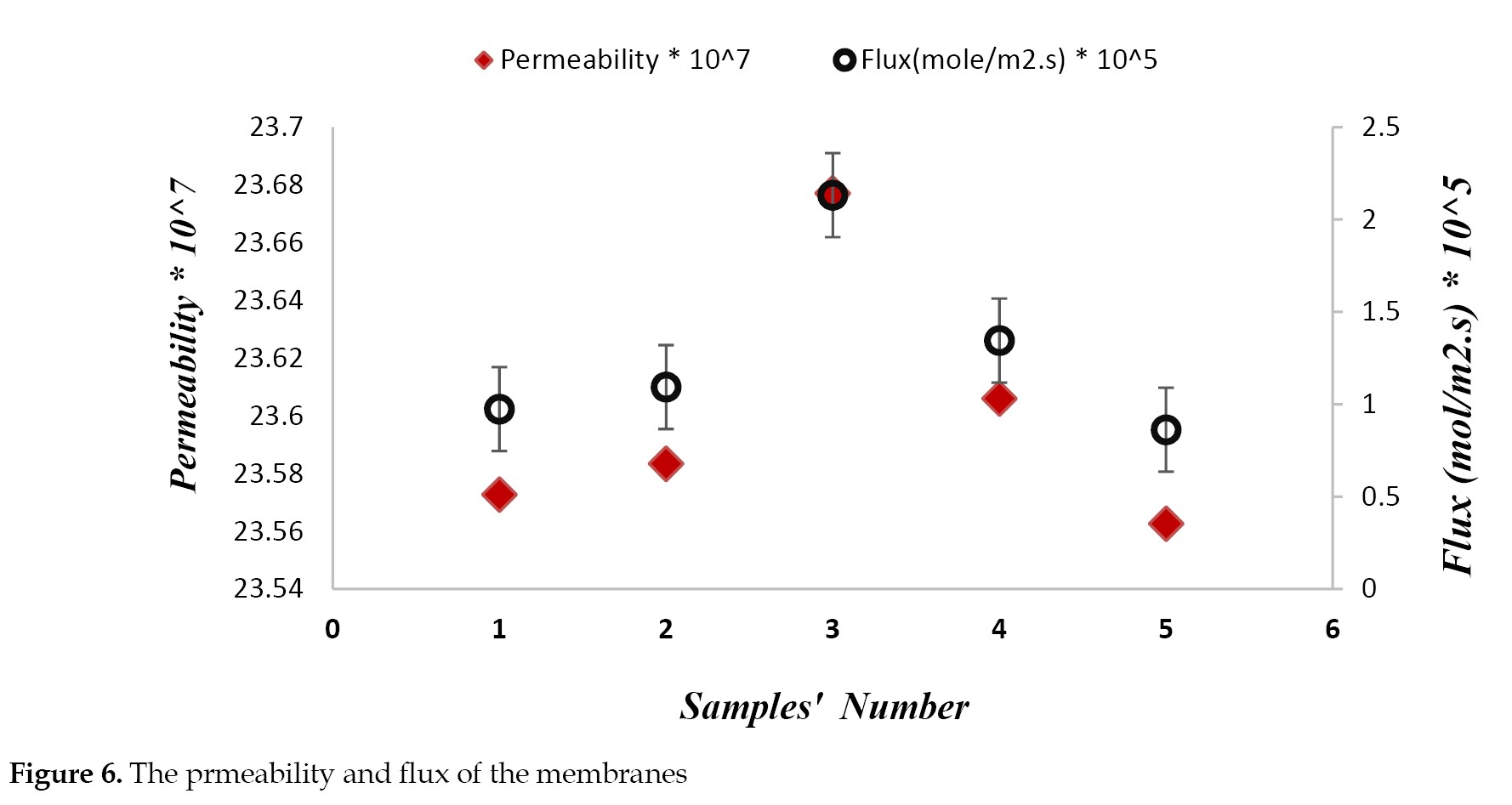
This may be due to the hydrophilic properties of PDA and controlled interspaces between the accumulated PDA and nanoaggregates [
21,
24]. Furthure, the membrane ionic permeability and flux increased with a rise in the CNF nanofibers’ loading ratio up to 0.07% in weight. This may be associated with the appropraite water content, and ionic pathways formation in the membranes. With greater increases in the nanofiber concentration from 0.07% to 0.3%, the ionic permeability and flux began to decline. This may be due to the lower percentage of water content. This event may also result from narrow channel formation restricting ion transport through the membranes. Moreover, this may also be due to nanofibers agglomeration with higher concentration of nanofibers in the casting solution, decreasing the membranes charge density.
Electrical resistance:
Figure 7 displays the samples’areal electrical resistance (ER).
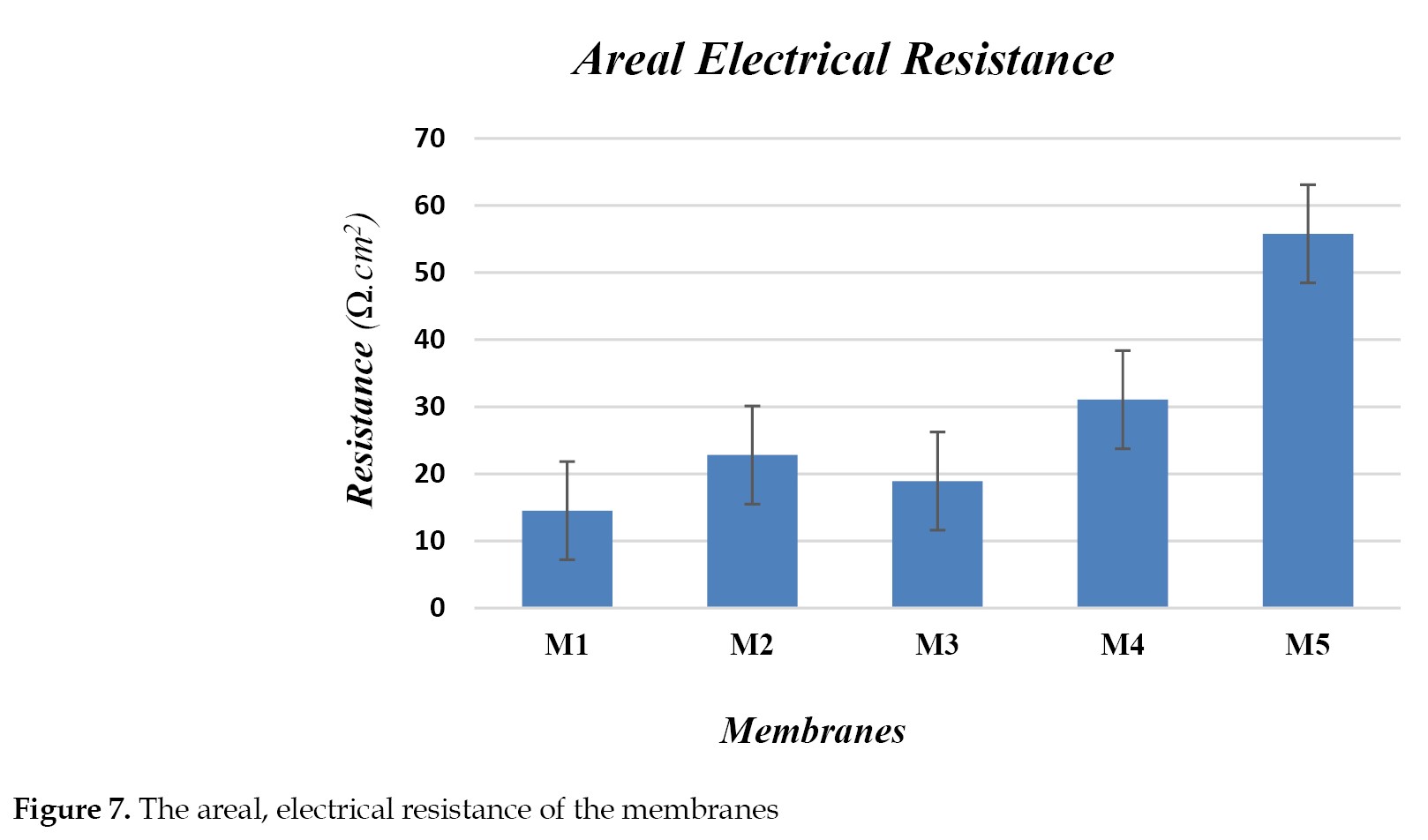
As seen in the Figure, the membranes surface modification with PDA plus the CNF incorporation to the surface up to 0.1% resulted in nearly a constant areal electrical resistance. This may be due to appropraite antifouling potential and the greater hydrophilicity of PDA [
38]. With a greater increase in CNF nanofibers concentration in the modified layers up to 0.3%, the membranes’ electrical resistance increases clearly, likely due to restriction against the oinic transport. The rise in the samples heterogeneity in the presence of added nanofibers increased the ionic transport but decreased the resistance (up to M3). With further increases in the CNF loading ratio and decline in the water content (
Table 2), the porous spots can be surrounded by nanofibers, hence a decline in the ionic transport. Therefore, under such conditions, the electrical resistance rises with further increases in the nanofibers concentration in the modified layers.
Conclusions
The surface hydrophilicity of the samples increased in the presence of PDA together with PVC-PDA-CNF. The modified membrane developed in this study offers a greater sodium flux compared to that achieved by unmodified membranes. Also the modified membranes had appropriate water content, higher permeability and ion exchange capacity. Application of membranes with the surfaces modified with PDA can improve the elimination efficiency of toxic heavy metal ions from wastewaters. In general, PVC-PDA-DLMs in lower loading ratio of CNF are desireable for water desalination and removal of heavy metal ions from wastewaters.
Name of the institution where the work was done: Department of Chemical Engineering, Faculty of Engineering, Arak University, Arak, Iran.
Ethical Considerations
Compliance with ethical guidelines
There were no animals or humans used in this study; therefore, ethical considerations were not applicable.
Funding
This study was funded by Arak University.
Authors' contributions
Conceptualization and supervision: Ezzatollah Joudaki and Sayedmohsen Hosseini; Investigation, writing - original draft: Amin Seidypoor and Samaneh Bandehali; Methodology, data analysis, writing - review & editing: All authors; Data collection: Amin Seidypoor; Funding acquisition and Resources: Amin Seidypoor. Ezzatollah Joudaki.
Conflict of interest
The authors declared no conflict of interests.
Acknowledgements
The authors gratefully acknowledge the Department of Chemical Engineering, Faculty of Engineering, Arak University, for providing the required laboratory equipment and space toward the implementation of this research.
References
- Peñate B, García-Rodríguez L. Current trends and future prospects in the design of seawater reverse osmosis desalination technology. Desalination. 2012; 284:1-8. [DOI:10.1016/j.desal.2011.09.010]
- Santhosh C, Velmurugan V, Jacob G, Jeong SK, Grace AN, Bhatnagar A. Role of nanomaterials in water treatment applications: A review. Chemical Engineering Journal. 2016; 306:1116-37. [DOI:10.1016/j.cej.2016.08.053]
- Chung TS, Jiang LY, Li Y, Kulprathipanja S. Mixed matrix membranes (MMMs) comprising organic polymers with dispersed inorganic fillers for gas separation. Progress in Polymer Science. 2007; 32(4):483-507. [DOI:10.1016/j.progpolymsci.2007.01.008]
- Yu L, Ruan SC, Xu XT, Zou RJ, Hu J. One-dimensional nanomaterial-as-sembled macroscopic membranes for water treatment. Nano Today. 2017; 17:79-95. [DOI:10.1016/j.nantod.2017.10.012]
- Gin DL, Noble RD, Designing the next generation of chemical separation mem-branes. Science. 2011; 332(6030):674-6. [DOI:10.1126/science.1203771] [PMID]
- Logan BE, Elimelech M. Membrane-based processes for sustainable power gen-eration using water. Nature. 2012; 488(7411):313-9. [DOI:10.1038/nature11477] [PMID]
- Jamaly S, Darwish NN, Ahmed I, Hasan SW. A short review on reverse osmosis pretreatment technologies. Desalination. 2014; 354:30-8. [DOI:10.1016/j.desal.2014.09.017]
- Pérez-González A, Urtiaga AM, Ibáñez R, Ortiz I. State of the art and review on the treatment technologies of water reverse osmosis concentrates. Water Research. 2012; 46(2):267-83. [DOI:10.1016/j.watres.2011.10.046] [PMID]
- Spiegler KS, El-Sayed YM. The energetics of desalination processes. Desalination. 2001; 134(1-3):109-28. [DOI:10.1016/S0011-9164(01)00121-7]
- Turek M. Cost effective electrodialytic seawater desalination. Desalination. 2003; 153(1-3):371-6. [DOI:10.1016/S0011-9164(02)01130-X]
- Wang Z, Luo Y, Yu P. Recovery of organic acids from waste salt solutions derived from the manufacture of cyclohexanone by electrodialysis. Journal of Membrane Science. 2006; 280(1-2):134-7. [DOI:10.1016/j.memsci.2006.01.015]
- Liu R, Wang Y, Wu G, Luo J, Wang S. Development of a selective electrodialysis for nutrient recovery and desalination during secondary effluent treatment. Chemical Engineering Journal. 2017; 322:224-33. [DOI:10.1016/j.cej.2017.03.149]
- Alabi A, Cseri L, Al-Hajaj A, Szekely G, Budde P, Zoua L. Electrostatically-coupled graphene oxide nanocomposite cation exchange membrane. Journal of Membrane Science. 2020; 594:117457. [DOI:10.1016/j.memsci.2019.117457]
- Alabi A, Al-Hajaj A, Cseri L, Szekely G, Budde P, Zoua L. Review of nanomaterials- assisted ion exchange membranes for electromembrane desalination. NPJ Clean Water. 2018; 1(1):1-22. [DOI:10.1038/s41545-018-0009-7]
- Hosseini SM, Madaeni SS, Khodabakhshi AR. Preparation and characterization of PC/SBR heterogeneous cation exchange membrane filled with carbon nano- tubes. Journal of Membrane Science. 2010; 362(1-2):550-9. [DOI:10.1016/j.memsci.2010.07.015]
- Klaysom C, Moon SH, Ladewig BP, Lu GM, Wang L. The influence of inorganic filler particle size on composite ion-exchange membranes for desalination. The Journal of Physical Chemistry C. 2011; 115(31):15124-32. [DOI:10.1021/jp112157z]
- Cseri L, Baugh J, Alabi A, Al-Hajaj A, Zou L, Dryfe RAW, et al. Graphene oxide-polybenzimidazolium nanocomposite anion exchange membranes for electrodialysis. Journal of Materials Chemistry A. 2018; 6(48):24728-39. [DOI:10.1039/C8TA09160A]
- Nemati M, Hosseini SM, Parvizian F, Rafiei N, Van der Bruggen B. Desalination and heavy metal ion removal from water by new ion exchange membrane modified by synthesized NiFe2O4/HAMPS nanocomposite. Ionics. 2019; 25:3847-57. [DOI:10.1007/s11581-019-02937-2]
- You X, Wu H, Su Y, Yuan J, Zhang R, Yu Q, et al. Precise nanopore tuning for high-throughput desalination membrane via codepostion of dopamine and multifunctional POSS. Journal of Materials Chemistry A. 2018; 27:1-33. [DOI:10.1039/C8TA03673J]
- Zhu J, Tian M, Zhang Y, Zhang H, Liu J. Fabrication of a novel ‘loose’ nanofiltration membrane by facile blending with Chitosan Monmorillonite nanosheets for dyes purification. Chemical Engineering Journal. 2015; 265:184-93. [DOI:10.1016/j.cej.2014.12.054]
- Xi ZY, Xu YY, Zhu LP, Wang Y, Zhu BK. A facile method of surface modification for hydrophobic polymer membranes based on the adhesive behavior of poly(DOPA) and poly(dopamine). Journal of Membrane Science. 2009; 327(1-2):244-53. [DOI:10.1016/j.memsci.2008.11.037]
- Kasemset S, Lee A, Miller DJ, Freeman BD, Sharma MM. Effect of polydopaminedeposition conditions on fouling resistance, physical properties, and permeation properties of reverse osmosis membranes in oil/water separation. Journal of Membrane Science. 2013; 425-426:208-16. [DOI:10.1016/j.memsci.2012.08.049]
- Lee H, Dellatore SM, Miller WM, Messersmith PB. Mussel-inspired surface chemistry for multifunctional coating. Science. 2017; 318(5849):426-30. [PMID] [PMCID]
- Wang J, Zhu J, Tsehaye MT, Li J, Dong G, Yuan S, et al. Hghi flux electroneutral loose nanofiltration membranes based on rapid dposition of polydopamine. Journal of Materials Chemistry A. 2017; 5(28):14847-57. [DOI:10.1039/C7TA02661G]
- Tiemblo P, Guzman J, Riande E, Mijangos C, Reinecke H. Effect of physical aging on the gas transport properties of PVC and PVC modified with pyridine groups. Polymer. 2001; 42(11):4817-23. [Link]
- Grafe T, Graham K. Polymeric nanofibers and nanofiber webs: A new class of nonwovens. International Nonwovens Journal. 2003; 12(1). [DOI:10.1177/1558925003os-1200113]
- Li X, Wang Z, Lu H, Zhao C, Na H, Zhao C. Electrochemical properties of sul-fonated PEEK used for ion exchange membranes. Journal of Membrane Science. 2005; 254(1-2):147-55. [DOI:10.1016/j.memsci.2004.12.051]
- Tanaka Y. Ion exchange membranes: Fundamentals and applications. Amsterdam: Elsevier; 2015. [Link]
- Kerres J, Cui W, Disson R, Neubrand W. Development and characterization of crosslinked ionomer membranes based upon sulfinated and sulfonated PSU Crosslinked PSU blend membranes by disproportionation of sulfinic acid groups. Journal of Membrane Science. 1998; 139(2):211-25. [DOI:10.1016/S0376-7388(97)00253-6]
- Nagarale R, Shahi VK, Thampy S, Rangarajan R. Studies on electrochemical characterization of polycarbonate and polysulfone based heterogeneous cation-ex-change membranes. Reactive and Functional Polymers. 2004; 61(1):131-8. [DOI:10.1016/j.reactfunctpolym.2004.04.007]
- Hunter RJ. Calculation of activity coefficient from Debye-Huckel theory. Journal of Chemical Education. 1966; 43(10):550. [DOI:10.1021/ed043p550]
- Xie Y, Yue L, Zheng Y, Zhao L, Liang C, He W, et al. The antibacterial stability of poly (dopamine) in-situ reduction and chelation nano-Ag based on bacterial cellulose network template. Applied Surface Science. 2019; 491:383-94. [DOI:10.1016/j.apsusc.2019.06.096]
- Ji YL, Qian WJ, An QF, Huang SH, Lee KR, Gao CJJ. Mussel-inspired zwitterionic dopamine nanoparticles as building blocks for constructing salt selective nanocomposite membranes. Journal of Membrane Science. 2019; 572:140-51. [DOI:10.1016/j.memsci.2018.11.019]
- Seidypoor A, Joudaki E, Hosseini SM, Bandehali S. Double-layer electrodialysis cation exchange membrane by introducing chitosan/TiO2 thin-film nanocomposite on PVC-based substrate for Cu removal from water. Ionics. 2022; 28:3037–48. [DOI:10.1007/s11581-022-04518-2]
- Cheng B, Li Z, Li Q, Ju J, Kang W, Naebe M. Development of smart poly (vinylidene fluoride)-graft-poly (acrylic acid) tree-like nanofiber membrane for pH-responsive oil/water separation. Journal of Membrane Science. 2017; 534:1-8. [DOI:10.1016/j.memsci.2017.03.053]
- Liu J, Shen X, Zhao Y, Chen L. Acryloylmorpholine-grafted PVDF membrane with improved protein fouling resistance. Industrial & Engineering Chemistry Research. 2013; 52(51):18392-400. [DOI:10.1021/ie403456n]
- Vrijenhoek EM, Hong S, Elimelech M. Influence of membrane surface properties on initial rate of colloidal fouling of reverse osmosis and nanofiltration membranes. Journal of Membrane Science. 2001; 188(1):115-28. [DOI:10.1016/S0376-7388(01)00376-3]
- Vaselbehagh M, Karkhanechi H, Mulyati S, Takagi R, Matsuyama H. Improved antifouling of anion-exchange membrane by polydopamine coating in electrodialysis process. Desalination. 2014; 332(1):126-33. [DOI:10.1016/j.desal.2013.10.031]
- Chakrabarty T, Singh AK, Shahi VK. Zwitterionic silica copolymer based crosslinked organic-inorganic hybrid polymer electrolyte membranes for fuel cell applications. RSC Advances. 2012; 2(5):1949-61. [DOI:10.1039/c1ra00228g]
- Geise GM, Paul DR, Freeman BD. Fundamental water and salt transport properties of polymeric materials. Progress in Polymer Science. 2014; 39(1):1-42. [DOI:10.1016/j.progpolymsci.2013.07.001]
- Bandehali S, Moghadassi A, Parvizian F, Zhang Y, Hosseini SM, Shen J. New mixed matrix PEI nanofiltration membrane decorated by glycidyl-POSS functionalized graphene oxide nanoplates with enhanced separation and antifouling behaviour: Heavy metal ions removal. Separation and Purification Technology. 2020; 242:116745. [DOI:10.1016/j.seppur.2020.116745]
- Zareei F, Hosseini SM. A new type of polyethersulfone based composite nanofiltration membrane decorated by cobalt ferrite-copper oxide nanoparticles with enhanced performance and antifouling property. Separation and Purification Technology. 2019; 226:48-58. [DOI:10.1016/j.seppur.2019.05.077]
- Hosseini SM, Sohrabnejad S, Nabiyouni G, Jashni E, Van der Bruggen B, Ahmadi A. Magnetic cation exchange membrane incorporated with cobalt ferrite nanoparticles for chromium ions removal via electrodialysis. Journal of Membrane Science. 2019; 583:292-300. [DOI:10.1016/j.memsci.2019.04.069]
- Bandehali S, Parvizian F, Moghadassi A, Hosseini SM. High water permeable PEI nanofiltration membrane modified by L-cysteine functionalized POSS nanoparticles with promoted antifouling/separation performance. Separation and Purification Technology. 2019; 237:116361. [DOI:10.1016/j.seppur.2019.116361]